Daniel Joseph Brotman, M.D.
- Director, Hospitalist Program, The Johns Hopkins Hospital
- Professor of Medicine
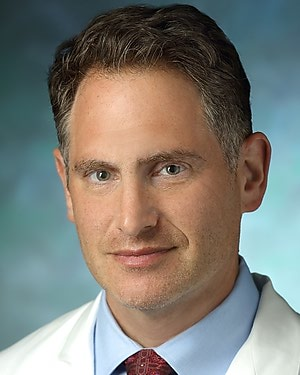
https://www.hopkinsmedicine.org/profiles/results/directory/profile/0000472/daniel-brotman
Each pit contains clusters of hairlike sensory cells whose cilia project into a gelatinous substance that is open to the water in which the animal swims spasms in intestines buy robaxin with american express. The purpose of lateral line organs in many animals is to sense vibrations or pressure changes in the water zyprexa spasms discount robaxin american express. Lateral line organs were lost as reptiles evolved spasms on left side of chest order robaxin paypal, but the exquisite mechanical sensitivity of hair cells was adopted and adapted for use in the structures of the inner ear that derived from the lateral line muscle relaxant 5859 discount robaxin 500mg with visa. The vestibular labyrinth includes two types of structures with different functions: the otolith organs muscle spasms 37 weeks pregnant order robaxin 500mg fast delivery, which detect the force of gravity and tilts of the head, and the semicircular canals, which are sensitive to head rotation. The ultimate purpose of each structure is to transmit mechanical energy, derived from head movement, to its hair cells. Each is sensitive to different kinds of movement not because their hair cells differ but because of the specialized structures within which the hair cells reside. A set of vestibular organs resides on each side of the head, mirror images of each other. The Otolith Organs the saccule and utricle detect changes of head angle, as well as linear acceleration of the head. When you tilt your head, the angle between your otolith organs and the direction of the force of gravity changes. Forces due to linear acceleration are the sort you encounter when you ride in an elevator or a car as it starts or stops. In contrast, when a car or elevator moves smoothly at constant velocity, acceleration is zero, so there is no force (apart from gravitational force). Each otolith organ contains a sensory epithelium called a macula, which is vertically oriented within the saccule and horizontally oriented within the utricle when the head is upright. Movements are transduced by hair cells in the maculae when the hair bundles are deflected. When the utricular macula is level (the head is straight), the cilia from the hair cells also stand straight. When the head and macula are tilted, gravity pulls the otoconia, which deform the gelatinous cap, and the cilia bend. When the angle of the head changes, or when the head accelerates, a force is exerted on the otoconia; this exerts a force in the same direction on the gelatinous cap, which moves slightly, and the cilia of the hair cells bend. The bending of hairs toward the kinocilium results in a depolarizing, excitatory receptor potential. If the hairs are bent perpendicular to their preferred direction, they barely respond. The head can tilt and move in any direction, but the hair cells of the utricle and saccule are oriented to transduce all of them effectively. On each macula, the direction preferences of the hair cells vary in a systematic way. Because of the mirror-image orientation of the saccule and utricle on each side of the head, when a given head movement excites hair cells on one side, it will tend to inhibit hair cells in the corresponding location on the other. Thus, any tilt or acceleration of the head will excite some hair cells, inhibit others, and have no effect on the rest. The central nervous system, by simultaneously using the information encoded by the full population of otolithic hair cells, can unambiguously interpret all possible linear movements. The Semicircular Canals the semicircular canals detect turning movements of the head, such as shaking your head from side to side or nodding up and down. Hair cells in the vicinity of an arrow are all polarized the same way; their stereocilia are all oriented so that bending them in the direction of the arrow depolarizes them. Angular acceleration is generated by sudden rotational movements, and it is the primary stimulus for the semicircular canals. The cilia project into the gelatinous cupula, which spans the lumen of the canal within the ampulla. All the hair cells in an ampulla have their kinocilia oriented in the same direction, which means that they all get excited or inhibited together. The semicircular canals are filled with endolymph, the same fluid that fills the scala media of the cochlea. Bending of the cilia occurs when the canal is suddenly rotated about its axis like a wheel; as the wall of the canal and the cupula begin to spin, the endolymph tends to stay behind because of inertia. This force bows the cupula, which bends the cilia, which (depending on the direction of the rotation) either excites or inhibits the release of neurotransmitter from the hair cells onto the vestibular nerve axons. Head rotation causes the excitation of hair cells in one horizontal semicircular canal and the inhibition of hair cells in the other. The graphs show that long-lasting head rotation leads to adaptation of the firing in vestibular axons. When rotation is stopped, the vestibular axons from each side begin firing again, but with opposite patterns of excitation and inhibition. This mechanism explains why you felt dizzy and unbalanced whenever, as a child, you stopped spinning your body like a top; your semicircular canals were temporarily sending the message that your body was still spinning, but in the opposite direction. Together, the three semicircular canals on one side of the head help sense all possible head rotation angles. Each member of a pair sits within the same orientation plane as its partner and responds to rotation about the same axis. Vestibular axons fire at high rates even at rest, so their activity can be driven either up or down depending on the direction of rotation. Central Vestibular Pathways and Vestibular Reflexes the central vestibular pathways coordinate and integrate information about head and body movement and use it to control the output of motor neurons that adjust head, eye, and body positions. The vestibular nuclei also receive inputs from other parts of the brain, including the cerebellum, and the visual and somatic sensory systems, thereby combining incoming vestibular information with data about the motor system and other sensory modalities. Axons from the semicircular canals project to the medial vestibular nucleus, which sends axons via the medial longitudinal fasciculus to excite motor neurons of trunk and neck muscles that orient the head. This pathway helps the head stay straight even as the body cavorts around below it. Similar to the other sensory systems, the vestibular system makes connections to the thalamus and then to the neocortex. At the cortical level, there is considerable integration of information about movements of the body, the eyes, and the visual scene. It is likely that the cortex continually maintains a representation of body position and orientation in space, which is essential for our perception of equilibrium and for planning and executing complex, coordinated movements. One very important function of the central vestibular system is to keep your eyes pointed in a particular direction, even while you are dancing like a fool. Recall that accurate vision requires the image to remain stable on the retinas despite movement of the head (see Chapter 9). Motor axons from the abducens nucleus in turn excite the lateral rectus muscle of the right eye. These pathways are active when the head suddenly turns to the left, causing the eyes to turn to the right. Excitatory connections are denoted by green plus signs; the inhibitory connection is denoted by a red minus sign. Vestibular Pathology the vestibular system can be damaged in a variety of ways; for example, high doses of antibiotics such as streptomycin can be toxic to hair cells. People with bilateral lesions of the vestibular labyrinths have enormous trouble fixating on visual targets as they move about. When people with vestibular disturbances cannot stabilize an image on their moving retinas, they may also experience the disconcerting feeling that the world is constantly moving around them. Compensatory adjustments come with time, as the brain learns to substitute more visual and proprioceptive cues to help guide smooth and accurate movements. These movement detectors are surrounded by three sets of inner ear structures that give them selectivity for three different kinds of mechanical energy: periodic waves of air pressure (sound), rotational forces (head turns), and linear forces (head tilt or acceleration). Except for the similarity in transduction, and the fact that the hair cells of both systems are located in the inner ear, the auditory and vestibular systems are quite different. The sound sensed by audition comes mainly from the external environment, while the vestibular system senses only the movements of itself. Auditory and vestibular pathways are entirely separate except perhaps at the highest levels of the cortex. Auditory information is often at the forefront of our consciousness, while vestibular sensation usually operates unnoticed to coordinate and calibrate our every movement. We have followed the auditory pathways from the ear to cerebral cortex and seen the ways in which information about sound is transformed. Variations in the density of air are converted to movements of the mechanical components of the middle and inner ear, which are transduced into neural responses. The structures of the ear and cochlea are highly specialized for the transduction of sound. However, this fact should not blind us to the considerable similarities between the organization of the auditory system and that of other sensory systems. In the visual system, the code in the photoreceptors is retinotopic; the activity of a given photoreceptor indicates light at a particular location. The receptors in the auditory system establish a spatial code that is tonotopic because of the unique properties of the cochlea. In each system, the retinotopy or tonotopy is preserved as signals are processed in secondary neurons, the thalamus, and finally in sensory cortex. The convergence of inputs from lower levels produces neurons at higher levels that have more complex response properties. Another example of increasing visual complexity is the convergence of inputs from the two eyes, which yields binocular neurons that are important for depth perception. Analogously, in the auditory system, input from the two ears is combined to create binaural neurons, which are used for horizontal sound localization. How is the conduction of sound to the cochlea facilitated by the ossicles of the middle ear Why is it impossible to predict the frequency of a sound simply by looking at which portion of the basilar membrane is the most deformed Why would the transduction process in hair cells fail if the stereocilia as well as the hair cell bodies were surrounded by perilymph If inner hair cells are primarily responsible for hearing, what is the function of outer hair cells What mechanisms function to localize sounds in the horizontal and vertical planes What symptoms would you expect to see in a person who had recently had a stroke affecting A1 unilaterally How does the severity of these symptoms compare with the effects of a unilateral stroke involving V1 What is the advantage of this arrangement compared to an arrangement of all the cells in the same direction Imagine a semicircular canal rotating in two different ways: around its axis (like a rolling coin) or end over end (like a flipped coin). How would you expect the functions of the otolith organs and the semicircular canals to change in the weightless environment of space The vestibular system: multimodal integration and encoding of self-motion for motor control. Somatic sensation enables our body to feel, to ache, to sense hot or chill, and to know what its parts are doing. It is sensitive to many kinds of stimuli: the pressure of objects against the skin, the position of joints and muscles, distension of the bladder, and the temperature of the limbs and of the brain itself. When stimuli become so strong that they may be damaging, somatic sensation is also responsible for the feeling that is most offensive, but vitally important-pain. The somatic sensory system is different from other sensory systems in two interesting ways. First, its receptors are distributed throughout the body rather than being concentrated at small, specialized locations. Second, because it responds to many different kinds of stimuli, we can think of it as a group of at least four senses rather than a single one: the senses of touch, temperature, pain, and body position. The somatic sensory system is really a catch-all name, a collective category for all the sensations that are not seeing, hearing, tasting, smelling, and the vestibular sense of balance. If something touches your finger, you can accurately gauge the place, pressure, sharpness, texture, and duration of the touch. If the touch moves from your hand to your wrist, and up your arm to your shoulder, you can track its speed and position. Assuming you are not looking, this information is described entirely by the activity of the sensory nerves in your limb. A single sensory receptor can encode stimulus features such as intensity, duration, position, and sometimes direction. In this chapter, we divide our discussion of somatic sensation into two main parts: the sense of touch and the sense of pain. As we shall see, these different categories depend on different receptors, different axonal pathways, and different regions of the brain. The sense of body position, also called proprioception, is discussed in Chapter 13, where we will explore how this type of somatic sensory information is used to control muscle reflexes. The two major types of skin are called hairy and glabrous (hairless), as exemplified by the backs and palms of your hands. Skin performs an essential protective function, and it prevents the evaporation of body fluids into the dry environment we live in.
Thus muscle relaxant pediatrics order robaxin 500mg with amex, it is highly desirable to exploit bioadhesive materials for the formulation of nanosystems to be retained in the cul-de-sac after topical administration spasms spasticity muscle buy cheapest robaxin. Erodible nanosystems are superior because the selferoding process of the hydrolyzable polymer exerts less harm on tissue [18 back spasms 4 weeks pregnant purchase robaxin 500 mg line,19] spasm order robaxin 500 mg fast delivery. Nevertheless spasms gelsemium semper buy discount robaxin on line, these drugs are often ineffective due to poor patient compliance, low penetration through the epithelial barrier, and unwanted side effects. In the field of cornea, nanomedicine research has particularly focused on imaging, prevention, and/or reduction of corneal opacities and neovascularization [20e24]. Advantages of Ocular Nanomedicines the advantages offered by nanotechnology-based ocular drug delivery over the conventional dosage forms cannot be overemphasized. In addition, some of the materials utilized for nanomedicines are not biodegradable; as a result, accumulation in the body (particularly in the eye tissues) over a long period of time would pose a threat to the eye. The stability of the drug in some of the nanobased formulations is not guaranteed after a prolonged period of time. Some bioadhesive polymers are associated with problems like blurred vision and formation of a veil in the corneal area, leading to loss of eyesight. The problem of particle growth after a prolonged period of time is also a challenge for nanomedicines, especially with nanosuspensions. The various approaches fall into two main categories: bioavailability improvement and controlled-release drug delivery. Improvement in bioavailability and the duration of action of ocular active drugs are based on maximizing corneal drug absorption and minimizing precorneal drug loss [29]. Tactical design of novel delivery systems would lead to sustained drug delivery, which may provide controlled and continuous delivery of ocular active drugs. Microparticulates (micromedicines) and nanoparticulates (nanomedicines) have been shown to improve the ophthalmic bioavailability of the drugs and to control the release of the ophthalmic drugs to the anterior segment of the eye. The nanomedicines investigated for ocular drug delivery include, among Disadvantages of Ocular Nanomedicines One of the disadvantages of nanotechnological-based drug delivery systems formulated for administration to the eye is the possibility of toxicity. The toxicity of nanoparticles in ocular tissues can be affected by many factors, including chemistry, size, dose, the time of assessment, and the biodistribution pattern of the particles in the eye. Even though many of the studies have focused on the efficacy of the formulations, recent studies assessed the toxicity based on histological evaluation, immunohistochemistry, inflammation, and neuronal toxicity [26]. These delivery systems have been shown to provide different degrees of bioavailability enhancement or controlled release of ocular active drugs. Particulates such as nanoparticles, nanocapsules, submicron emulsions, and nanosuspensions improved the bioavailability of ocularly applied drugs [30,31]. Colloidal systems, both in liquid form (eg, micro- and nanoemulsions) and in solid form (eg, nanoparticles), have excellent potential for interaction with the eye. Due to their size and the characteristics of their surface, they are taken up strongly by the cornea, forming a drug reservoir and thus improving the permanence of drugs at the aqueous and vitreous humor levels and enhancing efficacy in treating diseases of the anterior eye through targeting. Nanoparticles are nanometer-sized structures designed as drug carriers in which the active ingredient is dissolved, entrapped/encapsulated, or adsorbed. Different nanoparticles afford different drug release kinetics, capacities, and stability, and they represent promising drug carriers for ophthalmic applications. Nanoforms of ocular drugs can achieve sustained intravitreal therapeutic drug concentrations and thus significantly enhance the ocular bioavailability of many ocular drugs in comparison with normal aqueous eye solutions [32]. These particulate delivery systems can improve patient compliance and may reduce systemic side effects. Nanoparticles have been engineered from various synthetic and natural biocompatible polymers, but those derived from natural polymers that are biocompatible, biodegradable, nontoxic, and nonantigenic are favored. Nanoparticulates have submicron properties, such as high surface area and energy and movement of the particles in liquid media (Brownian motion). The surface charge of nanoparticles determines the performance of the nanoparticle system in the body [34]. The particle size of externally applied colloidal carriers influences absorption or permeation through the ocular barriers. Delivery of drugs to the posterior site of the eye by application of drug solution is very difficult. The possibility of drug-loaded nanoparticles to reach the posterior site of ocular tissues and deliver drugs at targeted sites at effective therapeutic concentration is very high for various disorders like age-related macular degeneration, retinitis, diabetic retinopathy, and corneal/conjunctival squamous cell carcinoma [35]. Polymer-Based Nanomedicines for the Eye Polymeric nanoparticles are colloidal carriers with diameters ranging from 10 to 1000 nm. They have been widely studied as topical ocular drug delivery systems because of their enhanced adherence to the ocular surface and their controlled release of drugs [36,37]. These systems allow a greater amount of design flexibility in terms of the size, surface charge, and composition to improve drug penetration, retention time, and sustain drug delivery. In addition, they can be formulated and administrated as eye drops, which makes them ideal candidates for the treatment of corneal diseases. Given that the surface of the ocular tissues (eg, cornea conjunctiva) is negatively charged, cationic colloidal nanoparticles are expected to confer better penetration potential through the ocular membranes and barriers. Nanoparticles Nanoparticles are roughly defined as particles with a diameter smaller than 1 mm, consisting of various biodegradable materials, such as natural or synthetic polymers, lipids, phospholipids, and even metals. These polymeric nanoparticles seemed to be devoid of any irritant effect on cornea, iris, and conjunctiva. Furthermore, the intravitreal injections of a suspension of polylactic acid micro- and nanospheres containing 1% adriamycin/doxorubicin were reported to provide sustained, first-order release for approximately 2 weeks. Chitosan, a deacetylated chitin, is a biodegradable, biocompatible, and nontoxic polymer whose nanoparticles have been demonstrated to penetrate effectively conjunctival and corneal epithelial cells. It is a promising ophthalmic vehicle because of its probable superior mucoadhesiveness caused by electrostatic interactions with the negative charges of the mucosal layers. Cyclosporine Aeloaded chitosan nanoparticles resulted in significantly higher corneal and conjunctival drug levels than those treated with a suspension of cyclosporin A in a chitosan aqueous solution or in water [46]. It has also been demonstrated that the amounts of fluorescent nanoparticles in cornea and conjunctiva were significantly higher than those of a control solution. Also, a higher retention of chitosan nanoparticles in the conjunctiva compared with in the cornea was observed [47]. Nanosuspensions Nanosuspensions consist of pure, poorly watersoluble drugs suspended in an appropriate dispersion medium. Nanosuspension technology can be better utilized for drug compounds that form crystals with high energy content, which renders them insoluble in either organic (lipophilic) or hydrophilic media. Suspensions of polymeric nanoparticles, which are prepared from inert polymeric resins, can be utilized as important drug delivery vehicles, capable of prolonging drug release and enhancing bioavailability. Since these carriers do not irritate cornea, iris, or conjunctiva, they act as an inert carrier for ophthalmic drugs [48]. The positive charge on the nanoparticle surface facilitates their adhesion to the corneal surface [49]. Thus, it can be accepted that the use of nanosuspensions in ophthalmic pharmaceutical formulations is an attractive research area, offering a great possibility to overcome the inherent difficulties associated with ocular drug delivery [50]. Nanosuspensions have a mean size around 100 nm and a positive charge; this make them suitable for ophthalmic applications. A sustained release was observed in vitro as well as in vivo, resulting in a doubled area under the curve compared with an aqueous solution [52]. Application of nanosuspensions as a noninvasive approach seems to be a safer controlled ocular delivery of antiinflammation agents for inhibition of uveitis symptoms. Nanocrystals Nanocrystals are nanoparticles composed of 100% drug without any matrix material, typically with a size range between 200 and 500 nm [33]. The physicochemical properties of nanocrystals depend on the type of stabilizerdthat is, nonionic surfactants (eg, polysorbates, poloxamines, and poloxamer) and ionic surfactants (eg, bile salts and alkyl-sulfonates). Drug nanocrystals can be considered as a universal formulation approach for delivery of poorly soluble drugs. The salient advantage of nanocrystals is the fact that they can be delivered through various administration routes, 330 25. Dendrimers Dendrimers are macromolecular compounds made up of a series of branches around an inner core. They are attractive systems for drug delivery because of their nanometer size range, ease of preparation and functionalization, and ability to display multiple copies of surface groups for biological recognition processes. As a result, they can be used as an effective vehicle for ophthalmic drug delivery [56]. These bioadhesive polymers, however, are associated with problems like blurred vision and formation of a veil in the corneal area, leading to loss of eyesight. So, greater possibilities can be explored by using dendrimers as ophthalmic drug delivery vehicles [57]. Dendrimers are composed of concentric, geometrically progressive layers created through radial amplification from a single, central initiator core molecule containing either three or four reactive sites such as ammonia or ethylene diamine. These nanoscale macromolecules are three-dimensional and highly branched monodispersed nanostructures that are obtained by an iterative sequence of reaction steps producing a precise, unique branching structure [58]. These nanostructures provide globular nanosystems of 1e100 nm depending on the molecular weight and number of generations. Due to the unique architecture of the nanoliposomes, they facilitate drug absorption through the corneal and conjunctival epithelial cells [62]. Liposomes are closed vesicles (small lipid vesicles) composed of a phospholipid bilayer, and water-soluble drugs can be incorporated into their aqueous phase, whereas lipid-soluble drugs can be incorporated into their lipid phase [63]. Because they are noncovalent aggregates, their lipid composition, size, and electric charge can be easily controlled [64,65]. Their modification, with surface polymers, carbohydrates, and antibodies, can be easily achieved to facilitate targeting [66]. Liposomes can hold various types of solutes with different properties and molecular weights, such as fat-soluble molecules [68] water-soluble molecules [69], and amphiphilic molecules [70]. Because of these characteristics, studies have been conducted on the intravitreal injection of drug-bearing liposomes and have demonstrated that the release of the drug can be controlled, the half-life of the drug inside the vitreous body can be prolonged, and the toxicity of the drug can be reduced [71e76]. Of these, positively charged nanostructures seem to be preferentially captured at the negatively charged corneal surface and slow down drug elimination by lacrimal flow [15,78]. Nanoprecipitation Method this can also be referred to as the solvent displacement method. The polymere drugesolvent mixture is then injected into an aqueous phase containing a surfactant or stabilizer. When the organic phase comes in contact with the aqueous phase, diffusion of the organic solvent into the aqueous phase leaves "less solvent" available for polymer dissolution, thereby causing the polymer and drug to precipitate out of the solution. This can be attributed to a difference in the selective affinities of the solvent for the polymer and water, leading to a displacement reaction that causes the polymer existing in the molecular state in the organic solvent to be precipitated as nanoparticles. Also, at the liquid interface exists a surface tension that, alongside solvent flow and diffusion, causes turbulence that gives rise to polymercontaining solvent droplets. Upon evaporation of the solvent, polymer precipitates containing the drug are formed in the nanosize range [82,83]. This method is suitable for hydrophobic drugs and has been used by several authors to successfully produce nanoparticles [84e86]. Microemulsions and Nanoemulsions Microemulsions have good tissue permeability because of the small size of the micelles and the presence of a surfactant among the components, and they have proven to be effective for ophthalmic drugs [79]. The instillation of microemulsions containing dexamethasone in the eyes of rabbits enhanced intraocular permeability [80]. Nanoemulsions are nanometer droplets made by the heterogeneous dispersions of two immiscible liquids (oil-in-water or water-in-oil) to provide a transparent ocular drug delivery system. They are unique as they can solubilize both hydrophobic and hydrophilic drugs to improve the stability, half-life, and therapeutic efficacy of the drug delivery. Moreover, they provide a large interfacial area compared to the small droplet size. For example, lecithins have been used as the major emulsifiers in the preparation of ocular nanoemulsions [20,81]. Emulsion Solvent Evaporation Method As the name implies, this involves two steps: emulsion formation, and solvent removal via evaporation. Frequently used organic solvents include ethylacetate, chloroform, and dichloromethane. However, toxicity has limited the use of chloroform and dichloromethane in recent times. The polymer and drug are first dissolved in a suitable quantity of the solvent to form the organic phase. Subsequently, both phases are mixed together and homogenized using an emulsifier or homogenizer (eg, Ultra Turrax homogenizer). Continuous stirring of the emulsion formed leads to an evaporation of the organic solvent. Removal of the organic solvent causes a precipitation of the drug-containing polymer, yielding nanoparticles, the size of which depends on factors such as surfactant concentration, homogenization speed, and time. Preparation methods of nanoparticles include the following: nanoprecipitation, emulsion/solvent evaporation, the double-emulsion solvent evaporation method, the salting-out method, ionic gelation, emulsification solvent diffusion, the dialysis method, the 332 25. This method is suitable for lipophilic drugs, but it is limited by the use of organic solvents that may be hazardous to humans and animals [87,88]. Double-Emulsion Solvent Evaporation Method this is a modification of the emulsion solvent evaporation method. Here, a multiple emulsion (w/o/w) is formed as a result of a double emulsification process. Usually, a hydrophilic drug is mixed with water and incorporated into the polymer-containing organic solvent. The emulsion thus formed is then added to a second aqueous phase (containing a surfactant) and stirred continuously to form a w/o/w emulsion. This method is suitable for preparing hydrophilic drugs and has been successfully used by several researchers to prepare such drugs [89e92]. Salting-Out Method In this method, electrolytes with suitable solubility in water are used. When added to the organic solvente water mixture, they precipitate the organic solvent from the water mixture, hence the term salting out. This is then mixed with an aqueous solution containing a stabilizer and the salting-out agent (eg, magnesium chloride, calcium chloride, or sucrose) [93,94]. Ionic Gelation Method Here, biodegradable polymers like chitosan, sodium alginate, and gelatin are used [94].
Cheap robaxin 500mg on-line. Horse Balsam | Best Workout Partner | Pre-Workout Gel | Herbal Balm | Muscle Relaxant Gel.
Pain (and temperature) information from the face and head takes a path to the thalamus that is analogous to the spinal path muscle relaxant nerve stimulator purchase 500mg robaxin visa. The small-diameter fibers in the trigeminal nerve synapse first on second-order sensory neurons in the spinal trigeminal nucleus of the brain stem spasms film buy discount robaxin 500mg on-line. The axons of these cells cross and ascend to the thalamus in the trigeminal lemniscus muscle relaxant trade names order robaxin 500mg. In addition to the spinothalamic and trigeminothalamic pathways muscle relaxant food buy robaxin from india, other closely related pain (and temperature) pathways send axons into a variety of structures at all levels of the brain stem muscle relaxant medicines buy 500mg robaxin with amex, before they reach the thalamus. Some of these pathways are particularly important in generating sensations of slow, burning, agonizing pain, while others trigger a more general state of behavioral arousal and alertness. The spinothalamic tract and trigeminal lemniscal axons synapse over a wider region of the thalamus than those of the medial lemniscus. From the thalamus, pain and temperature information is projected to various areas of the cerebral cortex. Depending on the concurrent level of nonpainful sensory input and the behavioral context, the same level of nociceptor activity can produce more pain or less pain. Understanding this modulation of pain is of great importance because it may offer new strategies for the treatment of chronic pain, a condition that afflicts up to 20% of the adult population. However, pain evoked by activity in nociceptors can also be reduced by simultaneous activity in low-threshold mechanoreceptors (A fibers). Presumably, this is why it feels good to rub the skin around your shin when you bruise it. This may also explain an electrical treatment for some kinds of chronic, intractable pain. Wires are taped to the skin surface, and pain is suppressed when the patient simply turns on an electrical stimulator designed to activate large-diameter sensory axons. Their gate theory of pain suggests that certain neurons of the dorsal horns, which project an axon up the spinothalamic tract, are excited by both large-diameter sensory axons and unmyelinated pain axons. By this arrangement, activity in the pain axon alone maximally excites the projection neuron, allowing nociceptive signals to rise to the brain. However, if the large mechanoreceptive axon fires concurrently, it activates the interneuron and suppresses nociceptive signals. Stories abound of soldiers, athletes, and torture victims who sustained horrible injuries but apparently felt no pain. Strong emotion, stress, or stoic determination can powerfully suppress feelings of pain. The relay of nociceptive signals by the projection neuron is gated by the activity of an inhibitory interneuron. Activity in the non-nociceptive mechanoreceptor can suppress, or close the "gate" on, nociceptive signals before they can proceed to the spinothalamic tract. The signs indicate excitatory synapses and the signs indicate inhibitory synapses. These medullary neurons in turn project axons down to the dorsal horns of the spinal cord, where they can effectively depress the activity of nociceptive neurons. Opium, its active narcotic ingredients, and their analogues-including morphine, codeine, and heroin-are used and abused widely today, in most cultures of the world. These drugs, and others with similar actions, are called opioids, and they produce profound analgesia when taken systemically (see Chapter 6). They can also produce mood changes, drowsiness, mental clouding, nausea, vomiting, and constipation. The 1970s brought the stunning discoveries that opioids act by binding tightly and specifically to several types of opioid receptors in the brain, and that the brain itself manufactures endogenous morphine-like substances, collectively called endorphins (see Box 6. Because this effect is prevented by administering the specific blocker of opioid receptors, naloxone, the injected drugs must have acted by binding to opioid receptors in those areas. Naloxone can also block the analgesic effects induced by electrically stimulating these areas. At the cellular level, endorphins exert multiple effects that include suppressing the release of glutamate from presynaptic terminals and inhibiting neurons by hyperpolarizing their postsynaptic membranes. In general, extensive systems of endorphin-containing neurons in the spinal cord and brain stem prevent the passage of nociceptive signals through the dorsal horn and into higher levels of the brain where the perception of pain is generated (Box 12. Surprisingly, the inert substance is often reported to have the effect that patients are told to expect from the drug. The term placebo is used to describe such substances (the word is from the Latin meaning, "I shall please"), and the phenomenon is called the placebo effect. A majority of patients suffering from postoperative pain have reported getting relief from an injection of sterile saline! The opioid receptor antagonist naloxone can block the analgesic effect of the placebo, just as it antagonizes the effects of morphine, a true analgesic. Apparently, the belief that the treatment will work can be enough to cause activation of the endogenous pain-relief systems of the brain. Thermoreceptors Because the rate of chemical reactions depends on temperature, the functioning of all cells is sensitive to temperature. However, thermoreceptors are neurons that are exquisitely sensitive to temperature because of specific membrane mechanisms. For example, we can perceive changes in our average skin temperature of as little as 0. Temperaturesensitive neurons clustered in the hypothalamus and the spinal cord are important in the physiological responses that maintain stable body temperature, but it is the thermoreceptors in the skin that apparently contribute to our perception of temperature. Some spots about 1 mm wide are especially sensitive to either hot or cold but not both. The fact that the locations of hot and cold sensitivity are different demonstrates that separate receptors encode them. Also, small areas of skin between the hot and cold spots are relatively insensitive to temperature. The sensitivity of a sensory neuron to a change in temperature depends on the type of ion channels the neuron expresses. As a rule, each thermoreceptive neuron appears to express only a single type of channel, thus explaining how different regions of skin can show distinctly different sensitivities to temperature. If such heat is applied to wide areas of skin, it is usually painful, but if the heat is restricted to small regions of skin innervated by a cold receptor, it can produce a paradoxical feeling of cold. As with mechanoreceptors, the responses of thermoreceptors adapt during long-duration stimuli. Notice that a return to the original warm skin temperature causes opposite responses-transient silence of the cold receptor and a burst of activity in the warm receptor-before both return to their steady, adapted rates. Notice the striking sensations of hot and cold that occur with each change, but also notice how transient the sensations are. With thermoreception, as with most other sensory systems, it is the sudden change in the quality of a stimulus that generates the most intense neural and perceptual responses. The responses of cold and warm receptors to a step reduction in skin temperature are shown. Both receptors are most responsive to sudden changes in temperature, but they adapt over several seconds. Cold receptors are coupled to A and C fibers, while warm receptors are coupled only to C fibers. As we learned previously, small-diameter axons synapse within the substantia gelatinosa of the dorsal horn. Thus, if the spinal cord is transected on one side, there will be a loss of temperature sensitivity (as well as pain) on the opposite side of the body, specifically in those regions of skin innervated by spinal segments below the cut. Different types of somatic sensory information are necessarily kept separate in the spinal nerves because each axon is connected to only one type of sensory receptor ending. Segregation of sensory types continues within the spinal cord and is largely maintained all the way to the cerebral cortex. In this way, the somatic sensory system repeats a theme common throughout the nervous system: Several flows of related, but distinct, information are passed in parallel through a series of neural structures. The mixing of these streams occurs along the way, but only judiciously, until higher levels of processing are reached in the cerebral cortex. We saw other examples of parallel processing of sensory information in the chemical senses, vision, and audition. Exactly how the parallel streams of sensory data are melded into perception, images, ideas, and memories remains the Holy Grail of neuroscience. Thus, the perception of any handled object involves the seamless coordination of all facets of somatic sensory information. The bird in hand is rounded, warm, soft, and light in weight; its heartbeat flutters against your fingertips; its claws scratch; and its textured wings brush against your palm. In the chapters that follow, we describe how the brain begins to use sensory information to plan and coordinate movement. Imagine rubbing your fingertips across a pane of smooth glass and then across a brick. As far as your somatic sensory system is concerned, what is different about the two surfaces What purpose is served by the encapsulations around some sensory nerve endings in the skin At what levels of the nervous system are all types of somatic sensory information represented on the contralateral side: the spinal cord, the medulla, the pons, the midbrain, the thalamus, and the cortex Imagine this experiment: Fill two buckets with water, one relatively cold and one hot. Put your left hand into the hot water, your right hand into the cold, and wait one minute. Transmitting pain and itch messages: a contemporary view of the spinal cord circuits that generate gate control. The importance of the motor system was summarized by the pioneering English neurophysiologist Charles Sherrington in the Linacre lecture of 1924: "To move things is all that mankind can do. Behavior requires the coordinated action of various combinations of almost 700 muscles in a changing and often unpredictable environment. Have you heard the expression "running around like a chicken with its head cut off" It comes from the observation that some complex patterns of behavior (running around a barnyard, at least briefly) can be generated without the participation of the brain. There is a considerable amount of circuitry within the spinal cord for the coordinated control of movements, particularly stereotyped (repetitive) ones such as those associated with locomotion. This point was established early in this century by Sherrington and his English contemporary Thomas Graham Brown, who showed that rhythmic movements could be elicited in the hind legs of cats and dogs long after their spinal cords had been severed from the rest of the central nervous system. In this chapter, we will explore the peripheral somatic motor system: the joints, skeletal muscles, and spinal motor neurons and interneurons, and how they communicate with each other. In Chapter 14, we will take a look at how the brain influences the activity of the spinal cord. Smooth muscle lines the digestive tract, arteries, and related structures and is innervated by nerve fibers from the autonomic nervous system (see Chapter 15). Smooth muscle plays a role in peristalsis (the movement of material through the intestines) and the control of blood pressure and blood flow. Cardiac muscle is heart muscle, which contracts rhythmically even in the absence of any innervation. Each skeletal muscle is enclosed in a connective tissue sheath that, at the ends of the muscle, forms the tendons. We focus our attention on this system here because it is under voluntary control and it generates behavior. This joint is formed where the humerus, the upper arm bone, is bound by fibrous ligaments to the radius and ulna, the bones of the lower arm. Movement in the direction that closes the knife is called flexion, and movement in the direction that opens the knife is called extension. Contraction of the biceps causes flexion of the elbow, and contraction of the triceps causes extension. Contraction of the extensor pulls the left end of the bone upward, causing the right end to pivot downward (extension). Two other muscles cause flexion at this joint, the biceps brachii and the coracobrachialis (which lies under the biceps). Together, these muscles are called flexors of the elbow joint, and, because the three muscles all work together, they are called synergists of one another. The two synergistic muscles that cause extension of the elbow joint are the triceps brachii and the anconeus; these two muscles are called extensors. Because the flexors and extensors pull on the joint in opposite directions, they are called antagonists to one another. Even the simple flexion of the elbow joint requires the coordinated contraction of the synergistic flexor muscles and the relaxation of the antagonistic extensor muscles. Relaxing the antagonists allows movements to be faster and more efficient because the muscles are not working against one another. Other terms to note about somatic musculature refer to the location of the joints they act on. The muscles that are responsible for movements of the trunk are called axial muscles; those that move the shoulder, elbow, pelvis, and knee are called proximal (or girdle) muscles; and those that move the hands, feet, and digits (fingers and toes) are called distal muscles. The axial musculature is very important for maintaining posture, the proximal musculature is critical for locomotion, and the distal musculature, particularly of the hands, is specialized for the manipulation of objects.
Starch is a complex carbohydrate spasms rectum robaxin 500mg on-line, specifically a polymer of glucose spasms to the right of belly button purchase generic robaxin on-line, the essential sugar in our bodies muscle relaxant drugs methocarbamol order cheap robaxin on-line. The knockout mice seemed to be indifferent to sugar spasms right side of back cheap robaxin 500mg online, as expected muscle relaxant 2 order generic robaxin from india, but they continued to seek out starchy foods. A Many people also love carbonated drinks such as soft drinks, club soda, or beer. As with fats, we can often feel carbonation as the fizziness vibrates the skin of the mouth and tongue. That is at least part of the answer, but how do we discriminate between simple sourness and carbonation Carbonation sensation may require the proper combination of sour taste and tingling somatic sensation. People may not love calcium, but they certainly need it for healthy bones, brains, and all other organs. Many animals seem to find calcium salts very tasty when they have been deprived of calcium but reject them when they are sated with calcium. Oddly, an aversion to the taste of Ca2 in mice requires the T1R3 protein, and the taste of Ca2 in humans is attenuated by a substance that binds to T1R3. It is possible, although far from proven, that T1R3 is part of a dedicated calcium taste receptor. Wetness, like fattiness and carbonation, can be felt with the somatic sensory system. Distilled water given to humans has been variously described as sweet, salty, or bitter, depending on the test conditions. A specific taste receptor for water would seem to be a useful adaptation, and there is strong evidence for such receptors in insects. Facing a mirror, stick your tongue out and shine a flashlight on it, and you will see your papillae easily-small, rounded ones at the front and sides, and large ones in the back. Fungiform papillae are relatively large toward the back of the tongue and much smaller along the sides and tip. Microvilli at the apical end of the taste cells extend into the taste pore, the site where chemicals dissolved in saliva can interact directly with taste cells. Concentrations too low will not be tasted, but at some critical concentration, the stimulus will evoke a perception of taste; this is the threshold concentration. At concentrations just above threshold, most papillae tend to be sensitive to only one basic taste; there are sour-sensitive papillae and sweet-sensitive papillae, for example. When the concentrations of the taste stimuli are increased, however, most papillae become less selective. Whereas a papilla might have responded only to sweet when all stimuli were weak, it may also respond to sour and salt if they are made stronger. We now know that each papilla has multiple types of taste receptor cells and that each receptor type is specialized for a different category of taste. Taste Receptor Cells the chemically sensitive part of a taste receptor cell is its small membrane region, called the apical end, near the surface of the tongue. However, they do form synapses with the endings of the gustatory afferent axons near the bottom of the taste bud. Taste receptor cells also make both electrical and chemical synapses onto some of the basal cells; some basal cells synapse onto the sensory axons, and these may form a simple information-processing circuit within each taste bud. This process depends on an influence of the sensory nerve, because if the nerve is cut, the taste buds will degenerate. When an appropriate chemical activates a taste receptor cell, its membrane potential changes, usually by depolarizing. If the receptor potential is depolarizing and large enough, some taste receptor cells, like neurons, may fire action potentials. In any case, depolarization of the receptor membrane causes voltage-gated calcium channels to open; Ca2 enters the cytoplasm, triggering the release of transmitter molecules. Evidence from recent studies of mice suggests that most taste receptor cells respond primarily or even exclusively to just one of the five basic tastes. Some taste cells and many gustatory axons show multiple response preferences, however. Why will one cell respond only to a single chemical type while another responds to three or four categories of chemicals The answer is that the responses depend on the particular transduction mechanisms present in each cell. The nervous system has myriad transduction mechanisms that make it sensitive to chemicals, pressures, sounds, and light. The nature of the transduction mechanism determines the specific sensitivity of a sensory system. Some sensory systems have a single basic type of receptor cell that uses one transduction mechanism. However, taste transduction involves several different processes, and each basic taste uses one or more of these mechanisms. Taste stimuli, or tastants, may (1) directly pass through ion channels (salt and sour), (2) bind to and block ion channels (sour), or (3) bind to G-protein-coupled receptors in the membrane that activate second messenger systems that, in turn, open ion channels (bitter, sweet, and umami). These are familiar processes, very similar to the basic signaling mechanisms present in all neurons and synapses, which were described in Chapters 4, 5, and 6. The prototypical salty chemical is table salt (NaCl), which, apart from water, is the major component of blood, the ocean, and chicken soup. The taste of salt is mostly the taste of the cation Na, but taste receptors use very different mechanisms to detect low and high concentrations of it. Amiloride is a diuretic (a drug that promotes urine production) used to treat some types of hypertension and heart disease. The amiloride-sensitive sodium channel is quite different from the voltage-gated sodium channel that generates action potentials; the taste channel is insensitive to voltage and generally stays open. When you sip chicken soup, the Na concentration rises outside the receptor cell, and the gradient for Na across the membrane is made steeper. Na then diffuses down its concentration gradient, which means it flows into the cell, and the resulting inward current causes the membrane to depolarize. This depolarization-the receptor potential-in turn causes voltage-gated sodium and calcium channels to open near the synaptic vesicles, triggering the release of neurotransmitter molecules onto the gustatory afferent axon. Animals avoid very high concentrations of NaCl and other salts, and humans usually report that they taste bad. It appears that high salt levels activate bitter and sour taste cells, which normally trigger avoidance behaviors. How very salty substances stimulate bitter and sour taste cells is still a mystery. For example, NaCl tastes saltier than Na acetate, apparently because the larger anion, acetate, inhibits the salty taste of the cation. Tastants can interact directly with ion channels either by passing through them (Na and H) or by blocking them (H blocking the potassium channel). The membrane voltage then influences calcium channels on the basal membrane, which in turn influence the intracellular [Ca2] and transmitter release. Sodium saccharin tastes sweet because the Na concentrations are far too low for us to taste the saltiness, and the saccharin potently activates sweetness receptors. The transduction processes underlying bitter, sweet, and umami tastes rely on two families of related taste receptor proteins called T1R and T2R. The various subtypes of T1R and T2R are all G-proteincoupled taste receptors that are very similar to the G-protein-coupled receptors that detect neurotransmitters. Bitter substances are detected by the 25 or so different types of T2R receptors in humans. Bitter receptors are poison detectors, and because we have so many, we can detect a vast array of different poisonous substances. Animals are not very good at telling different bitter tastants apart, however, probably because each bitter taste cell expresses many, and perhaps most, of the 25 bitter receptor proteins. Since each taste cell can send only one type of signal to its afferent nerve, a chemical that can bind to one of its 25 bitter receptors will trigger essentially the same response as a different chemical that binds to another of its bitter receptors. The important message the brain receives from its taste receptors is simply that a bitter chemical is "Bad! Bitter receptors use a second messenger pathway to carry their signal to the gustatory afferent axon. In fact, the bitter, sweet, and umami receptors all seem to use the same second messenger pathway to carry their signals to the afferent axons. The taste cells for bitter, sweet, and umami lack conventional transmitter-filled presynaptic vesicles. Sweet receptors resemble bitter receptors, in that they are all dimers of G-protein-coupled receptors. If either one of them is missing or mutated, an animal may not perceive sweetness at all. In fact, all species of cats and some other carnivores lack the genes encoding T1R2 and are indifferent to the taste of most molecules that we consider sweet. The reason is that bitter receptor proteins and sweet receptor proteins are expressed in different taste cells. Bitter taste cells and sweet taste cells, in turn, connect to different gustatory axons. The activity of different gustatory axons reflects the chemical sensitivities of the taste cells that drive them, so the messages about sweetness and bitterness are delivered to the central nervous system along different transmission lines. The transduction process for umami is identical to that for sweetness, with one exception. The sweet and umami receptors share the T1R3 protein, so it is the other T1R that determines whether the receptor is sensitive to amino acids or sweet tastants. When the gene encoding for the T1R1 protein is removed from mice, they are unable to taste glutamate and other amino acids, although they still demonstrate a sense for sweet chemicals and other tastants. Similar to other types of taste receptors, the genetics of different mammalian species lead to interesting taste preferences and deficits. Most bats, for example, do not have a functioning T1R1 receptor and presumably cannot taste amino acids. The ancestors of bats presumably did have umami and sweet receptors; why they were lost is unknown. Once again, the taste cells selectively express only one class of taste receptor protein. There are umami-specific taste cells, just as there are sweet-specific taste cells and bitter-specific taste cells. The gustatory axons they stimulate are, in turn, delivering messages of umami, sweetness, or bitterness to the brain. Three cranial nerves carry primary gustatory axons and bring taste information to the brain. The regions around the throat, including the glottis, epiglottis, and pharynx, send taste axons to a branch of cranial nerve X, the vagus nerve. Gustatory nucleus axons synapse on neurons of the thalamus, which project to primary gustatory cortex in regions of the postcentral gyrus and insular cortex. The path to the neocortex via the thalamus is a common one for sensory information. The taste pathways to the thalamus and cortex are primarily ipsilateral to the cranial nerves that supply them. Gustation is important to basic behaviors such as the control of feeding and digestion, both of which involve additional taste pathways. Gustatory nucleus cells project to a variety of brain stem regions, largely in the medulla, that are involved in swallowing, salivation, gagging, vomiting, and basic physiological functions such as digestion and respiration. In addition, gustatory information is distributed to the hypothalamus and related parts of the basal telencephalon (structures of the limbic system; see Chapter 18). These structures seem to be involved in the palatability of foods and the forces that motivate us to eat (Box 8. Localized lesions of the hypothalamus or amygdala, a nucleus of the basal telencephalon, can cause an animal to either chronically overeat or ignore food, or alter its preferences for food types. Within an hour he became nauseated, vomited, and had a most unpleasant bus ride home. Sadly, for years afterward, he could not even imagine eating another fried clam, and the smell of them alone was repulsive. It did not affect his enjoyment of other foods, and he felt no prejudice against amusement park rides, buses, or the friends he had been with the day he got sick. By the time the author reached his thirties, he could happily dine on fried clams again. He also read about research that John Garcia, working at Harvard Medical School, had done just about the same time as the original bad-clam experience. Garcia fed rats a sweet liquid, and, in some cases, he then gave them a drug that made them briefly feel ill. After even one such trial, rats that had received the drug avoided the sweet stimulus forever. W Extensive research has shown that flavor aversion learning results in a particularly robust form of associative memory. It is most effective for food stimuli (taste and smell both contribute); it requires remarkably little experience (as little as one trial); and it can last a very long time-more than 50 years in some people! And learning occurs even when there is a very long delay between the food (the conditioned stimulus) and the nausea (the unconditioned stimulus). For modern humans, this memory mechanism can backfire; many perfectly good fried clams have remained uneaten.
References
- Liem MK, Lesnik Oberstein SA, et al. MRI correlates of cognitive decline in CADASIL: a 7-year follow-up study. Neurology 2009; 72:143.
- Kant, A.K. and B.I. Graubard, Energy density of diets reported by American adults: Association with food group intake, nutrient intake, and body weight. Int. J. Obes. (Lond.), 2005.
- Posner JB. Back pain and epidural spinal cord compression. Med Clin North Am 1987;71(2):185-205.
- Powers WJ, Martin WR, Herscovitch P, et al. Extracranialintracranial bypass surgery: hemodynamic and metabolic effects. Neurology 1984;34:1168-74.
- Handke M, Harloff A, Olschewski M, et al. Patent foramen ovale and cryptogenic stroke in older patients. New Engl J Med 2007;357:2262-8.
- Frankel TL, Chang AE, Wong SL. Surgical options for localized and advanced gastrointestinal stromal tumors. J Surg Oncol 2011;104(8):882-887.
- Skidmore R, Woodcock JP. Physiological interpretation of Doppler - shift waveforms. II. Validation of the Laplace transform method for characterization of the common femoral blood - velocity/time waveform. U ltrasound Med Biol 1980; 6:219.
- Snyder EL, Hezzey A, Cooper-Smith M, et al: Effect of microaggregate blood filtration on platelet concentrates in vitro. Transfusion 21:427-434, 1981.